Last Updated on May 17, 2025
The global energy transition hinges on the ability to store renewable energy efficiently and affordably. While lithium-ion (Li-ion) batteries dominate today’s market, their limitations in cost, safety, and scalability for grid applications have spurred innovation in alternative materials and technologies.
By 2024, the cumulative capacity of Battery Energy Storage Systems (BESS) reached 150 GW / 363 GWh, with a market value exceeding $90 billion. Yet, as demand surges for long-duration storage and safer solutions, researchers and companies are exploring novel chemistries and designs. This article examines six emerging and mature technologies reshaping large-scale energy storage, their scientific foundations, and their pros and cons.
Why Lithium-Ion Falls Short for Grid-Scale Storage
Li-ion batteries, while revolutionary for portable electronics and electric vehicles, face critical challenges in grid applications:
1. Cost Limitations: Though Li-ion BESS costs dropped to $165/kWh in 2024 (40% lower than in 2023), they remain uneconomical for durations exceeding 8 hours.
2. Safety Risks: Flammable electrolytes and thermal runaway risks complicate deployment in industrial settings.
3. Resource Constraints: Lithium and cobalt shortages and geopolitical mining concerns threaten long-term scalability.
4. Performance Gaps: Li-ion struggles with ultra-long-duration storage (e.g., multi-day grid resilience), prompting demand for alternatives.
Emerging and Mature Alternatives for Grid-Scale Storage
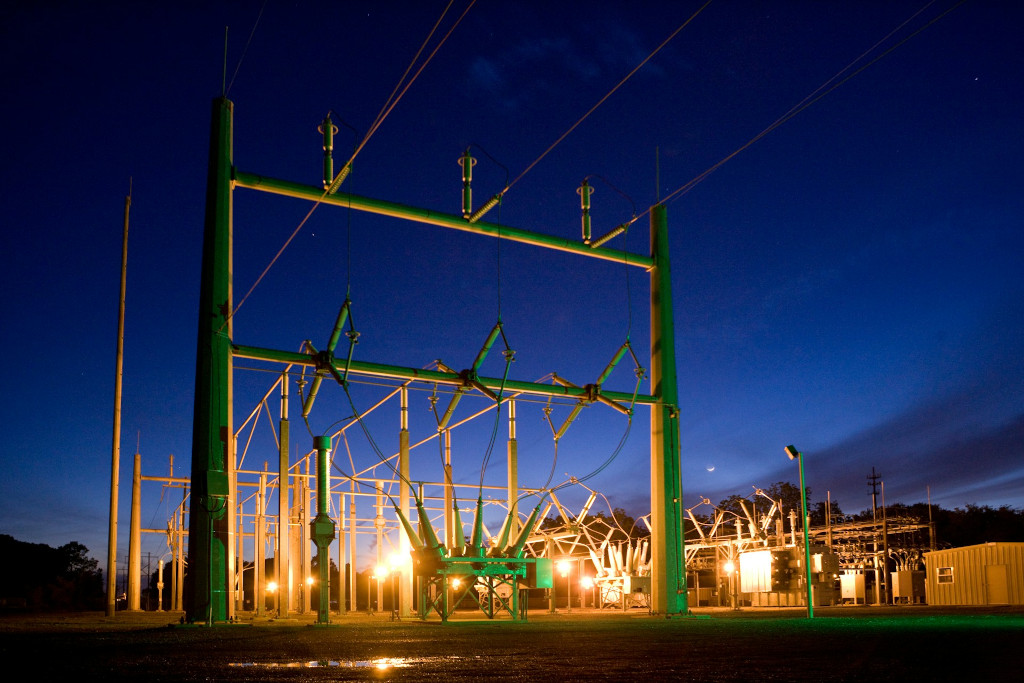
1. Sodium-ion batteries (SIBs)
Sodium-ion batteries are a type of rechargeable battery that uses sodium ions (Na⁺) instead of lithium ions (Li⁺) to store and release energy. They work similarly to lithium-ion batteries but are often cheaper because sodium is more abundant and less expensive than lithium.
Here’s how they work:
- Charging: When you charge the battery, sodium ions move from the cathode (positive electrode) through an electrolyte to the anode (negative electrode), where they are stored.
- Discharging: When you use the battery, the sodium ions move back to the cathode through the electrolyte, releasing energy that powers your device.
Sodium-ion batteries are safer and more environmentally friendly but currently store less energy than lithium-ion batteries.
Science: Sodium-ion chemistry mimics Li-ion but uses abundant sodium, reducing material costs by 20–30%. Recent advances in cathode materials—such as layered transition metal oxides and Prussian blue analogues (PBAs)—have improved energy density and cycle life.
Progress:
- A 2024 alkaline aqueous sodium-ion battery with a Mn-based PBA cathode achieved 13,000 cycles at 10 C and 88.9 Wh/kg energy density, rivaling Li-ion performance.
- China deployed a 50 MW/100 MWh sodium-ion grid system in Hubei Province, signaling commercial viability.
Pros:
- Lower cost ($66/kWh in China for large-scale systems).
- Compatibility with existing Li-ion manufacturing infrastructure.
Cons:
- Energy density (~120–160 Wh/kg) lags behind Li-ion (~250 Wh/kg).
2. Zinc-Based Batteries
Zinc-based batteries are a class of energy storage systems that utilize zinc as a primary component in their electrochemical reactions. These batteries typically consist of a zinc anode, a cathode (often made of materials like manganese dioxide or air), and an electrolyte that facilitates ion movement.
During discharge, zinc atoms oxidize at the anode, releasing electrons that power external devices, while zinc ions migrate through the electrolyte to the cathode. During charging, the process reverses, restoring the zinc anode.
Zinc-based batteries are promising for grid-scale energy storage due to their cost-effectiveness, safety, and abundance of zinc as a raw material.
Science: Zinc’s stability in aqueous electrolytes enables inherently safe, low-cost systems. Technologies include zinc-ion (Zn-ion) and zinc-bromine flow batteries (Zn-Br).
Progress:
Zn-Br flow batteries (e.g., Redflow’s projects) avoid critical minerals, instead they use recyclable zinc and bromine. Over 270 deployments highlight their suitability for off-grid and industrial applications.
Zn-ion batteries face challenges like dendrite formation but offer potential for <$50/kWh systems.
Pros:
- Non-flammable electrolytes ideal for high-heat environments (e.g., steel plants).
- Abundant materials reduce geopolitical risks.
Cons:
- Lower energy density (~50–100 Wh/kg) and limited cycle life (~1,000 cycles).
3. Flow Batteries
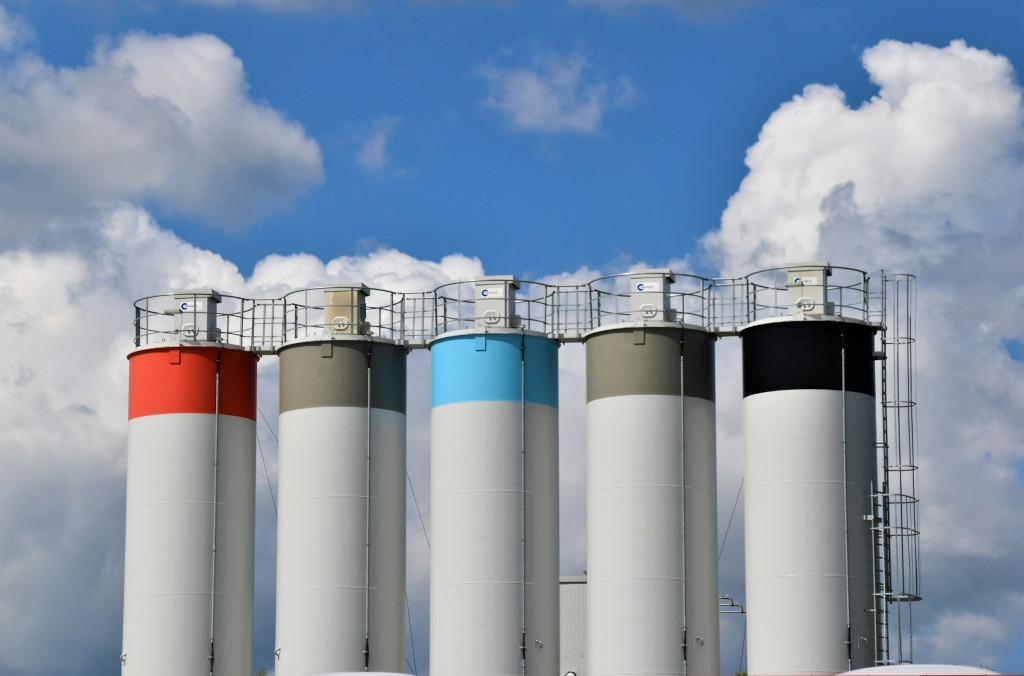
Flow batteries are rechargeable batteries that store energy in liquid electrolytes contained in external tanks. They consist of two electrolyte solutions, one positive and one negative, which flow through a cell stack separated by a membrane.
During charging, electrical energy drives chemical reactions that store energy in the electrolytes. During discharge, the process reverses, releasing energy as the electrolytes flow back through the cell stack.
Flow batteries are well-suited for grid-scale storage due to their scalability, long cycle life, and ability to decouple power and energy capacity.
The large external tanks allow for cost-effective scaling of energy storage duration, making them ideal for storing renewable energy over extended periods. Additionally, they have minimal degradation over thousands of cycles and are safer than some other battery types due to their non-flammable electrolytes.
While they have lower energy density compared to solid-state batteries, their durability and flexibility make them a promising solution for stabilizing grids with high renewable energy penetration.
Science: Flow batteries store energy in liquid electrolytes, enabling decoupled power and energy capacity. Key variants include vanadium, zinc-bromine, and soluble lead flow batteries (SLFB).
Progress:
Vanadium redox flow (VRFB): Mature but expensive ($300–600/kWh) due to vanadium costs.
SLFB: The University of Southampton’s design uses recycled lead-acid components, achieving non-flammable, circular manufacturing. SoLead Energy, its commercial spin-off, aims for grid pilots by 2026.
Pros:
- Scalable duration (4–12+ hours) and 20,000+ cycle lifespans.
- Minimal degradation compared to solid-electrode batteries.
Cons:
- Lower energy density (~25 Wh/kg) and complex system integration.
4. Metal-Air Batteries
Metal-air batteries are a type of battery that generates electricity by reacting a metal with oxygen from the air. They are lightweight and have high energy density, making them promising for applications like electric vehicles and portable electronics.
The metal (e.g., zinc, aluminum, or lithium) acts as the anode, while oxygen from the air serves as the cathode, reducing the need for heavy internal materials.
Science: These batteries use oxygen from the air as a cathode reactant, offering ultra-high theoretical energy density (e.g., lithium-air: ~3,500 Wh/kg).
Progress:
Iron-air batteries (e.g., Form Energy’s pilot) target $20/kWh for 100-hour storage, leveraging rusting/reversal cycles.
Zinc-air systems face challenges in rechargeability but show promise for rural electrification.
Pros:
- Exceptionally low material costs and long discharge times.
Cons:
- Low round-trip efficiency (~50%) and slow commercialization.
5. Lithium Iron Phosphate (LFP)
Mature Alternative: While still Li-ion, LFP cathodes mitigate some Li-ion drawbacks.
Lithium Iron Phosphate (LFP) batteries are a type of lithium-ion battery that uses lithium iron phosphate (LiFePO₄) as the cathode material and a graphite carbon electrode with a metallic backing as the anode. They are known for their safety, long cycle life, and thermal stability, making them a reliable choice for various applications.
Advantages:
- Safety: LFP batteries are more thermally and chemically stable than other lithium-ion chemistries, reducing the risk of overheating or fire, which is crucial for large-scale installations.
- Longevity: They have a long cycle life, often exceeding 2000-3000 charge-discharge cycles, which means they can be used for many years with minimal degradation.
- Cost-Effectiveness: While the initial cost might be higher, the long lifespan and low maintenance requirements make LFP batteries cost-effective over time.
- Durability: Dominates BESS deployments, with projects like California’s 821 MW/3,280 MWh Edwards & Sanborn facility.
Limitations:
- Lower energy density (~150 Wh/kg) compared to NMC Li-ion.
The Road Ahead
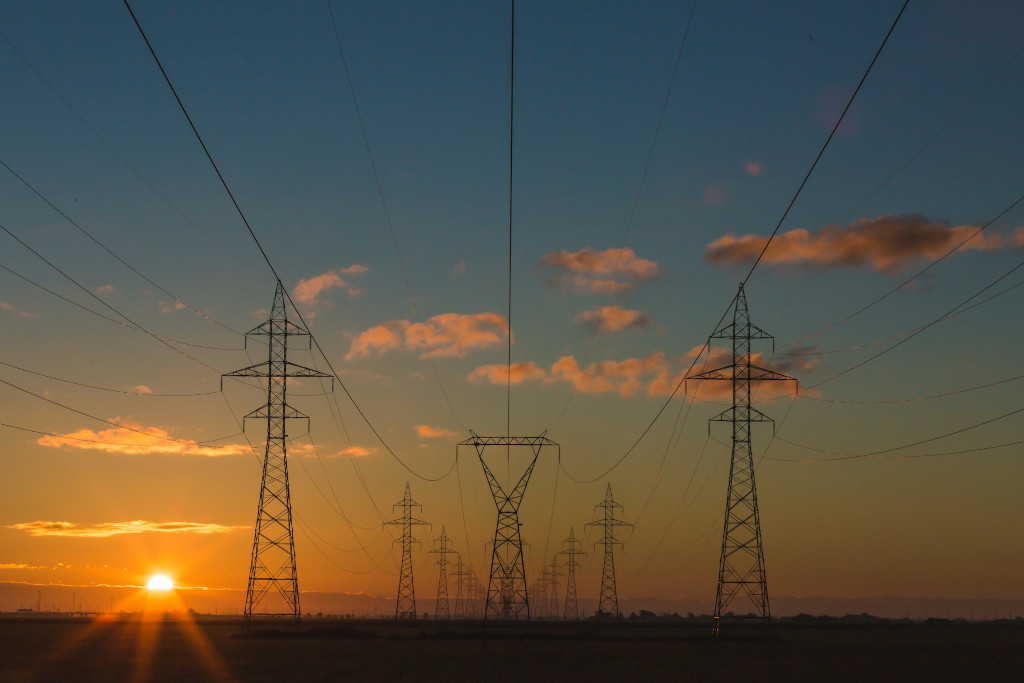
The race for grid-scale storage is accelerating, driven by climate goals and rising AI/data center energy demands (projected to triple US power consumption by 2030). While no single technology will dominate, hybrid systems combining sodium-ion for daily cycling, flow batteries for long-duration needs, and LFP for peak shaving could optimize grid resilience.
Innovations like PBAs and SLFBs highlight the potential for circular, sustainable designs.
Key Challenges Remain:
- Standardizing definitions for “long-duration” storage (4–100+ hours).
- Scaling manufacturing and improving supply chains for sodium and zinc.
- Regulatory support to phase out peaker plants (63 million Americans live near these polluting facilities).
As the International Energy Agency notes, grid storage must expand from 200 GW in 2023 to 5 TW by 2050 for net-zero targets. The 2020s, dubbed the “Decade of Energy Storage,” are poised to redefine how we power the world—one breakthrough chemistry at a time.